Antioxidant and anticancer activity of Artemisia princeps var. orientalis extract in HepG2 and Hep3B hepatocellular carcinoma cells
Introduction
An imbalance of oxidative stress could cause a condition in which cellular antioxidant defenses are insufficient to maintain the levels of oxidants below a risk threshold. An accumulation of reactive oxygen species (ROS) such as superoxide (O2.–, OOH.), hydroxyl (OH.), and peroxyl (ROOH.) radicals, reactive nitrogen species (RNS), and sulfur-centered radicals, can cause chronic diseases such as cancer, diabetes, and cardiovascular conditions.
It has recently been suggested that antioxidative molecules, which are widely distributed in most of the plants, could be very beneficial to human health in many ways. Plant antioxidants limit oxidative stress by scavenging free radicals, inhibiting oxidation, and activating enzymes of the antioxidant defense system. Therefore, increased consumption of herb plants, which might play important roles in the prevention of oxidative stress associated disorders and the maintenance of good health, has been recommended.
Artemisia princeps var. orientalis belongs to the Asteraceae family of plants, which consists of more than 500 species that are widely distributed.
Bioactive constituents of Artemisia spp. have been investigated in several studies, and some of them, such as terpenoids, flavonoids, coumarins, glycosides, sterols, and polyacetylenes, have been isolated from A. princeps (1-3). Previous studies have shown that these bioactive constituents have antimalarial, antiviral, antioxidant, and anticancer effects (4,5).
Although many people have broad experience in using traditional plant-derived product (drugs and supplements) for their health, the molecular mechanisms underlying the effects of A. princeps var. orientalis have not been studied. Although A. princeps var. orientalis may have great possibilities based on its use in home remedies, few studies have been reported (6). In the present study, we evaluated the antioxidant and anticancer activity of Artemisia princeps in human hepatoma HepG2 and Hep3B cells.
Materials and methods
Cells cultures and preparation of plant methanol extract
Hepatocellular cancer HepG2 and Hep3B cells were purchased from the Korean Cell Line Bank (KCLB, Korea) and routinely maintained in minimum essential medium [MEM, Invitrogen (Molecular Probes), Gibco, CA, USA], supplemented with 10% fetal bovine serum (FBS) and antibiotics (50 U/mL penicillin and 50 µg/mL streptomycin, Gibco) at 37 °C in a humidified atmosphere containing 5% CO2.
Methanol extract of A. princeps var. orientalis (APME) was supplied by Herbarium of the Korea Research Institute of Bioscience & Biotechnology (KRIBB). Taxonomical identification was confirmed by a botanist and voucher specimen (KRIB0000159) stored at the KRIBB Herbarium. APME was diluted in dimethyl sulfoxide (DMSO) to 10 mg/mL just before use.
Determination of antioxidant activity
To evaluate the antioxidant activity of APME, ROS and antioxidant enzymes, superoxide dismutase (SOD) and catalase were investigated in HepG2 cells exposed to APME (5, 100, and 200 µg/mL) for 24, 48, and 72 h. Cells were lysed by sonication and then centrifuged at 10,000 g for 30 min at 4 °C. The supernatant was used to determine the enzyme activity.
Detection of ROS
Production of intracellular ROS was measured by the fluorescence dyes, dichlorodihydro-fluorescein diacetate (H2DCFDA) and dihydrorhodamine 123 (DHR123, Molecular Probes, Eugene, OR). H2DCFDA is rapidly oxidized to the highly fluorescent dichlorofluorescein (DCF) and DHR123 is oxidized intracellularly to form the fluorescent compound rhodamine 123 (RH123) in the presence of ROS. Following the treatment of hydrogen peroxide (H2O2), cells were incubated with H2DCFDA or DHR123 in the absence or presence of up to 200 µg/mL APME. Fluorescence was determined at excitation wavelength of 529 nm/536 nm and emission wavelength of 503 nm/500 nm for H2DCFDA or DHR123, respectively, on a spectrofluorometer (Molecular devices, spectra max geminiXS, CA, USA).
Enzymes activities
The catalase activity was assayed by the method of Aebi (7). Briefly, a 20 µL sample was added to 480 µL of 10 mmol/L H2O2 in 20 mmol/L potassium phosphate buffer (pH 7.0) and incubated at 25 °C for 1.5 min. Initial reaction rate was measured from the decrease in absorbance at 240 nm. The catalase activity was calculated as nmol of H2O2 decomposed/(min.mg protein). SOD activity was assayed according to the pyrogallol autoxidation method of Marklund and Marklund (8). Briefly, a 250 µL sample was added to 750 µL reaction solution [50 mmol/L Tris buffer, pH 8.2, containing 1 mmol/L diethylenetriaminepentaacetic acid (DTPA), 10 µmol/L catalase and 200 µmol/L pyrogallol]. The change in absorbance at 420 nm was measured at 25 °C every 30 s for 5 min. SOD activity was defined as the quantity of enzyme that inhibited auto-oxidation of pyrogallol by 50% under experimental conditions. Protein concentration was determined by Bradford protein assay kit II (Bio-rad, Laboratories, CA, USA).
Determination of anticancerigen activity in hepatocarcinoma cells
Antiproliferative avtivity was investigated in HepG2 and Hep3B cells exposed to APME at a range of 5-200 µg/mL for 24, 48, and 72 h. Then, cell cycle distribution and apoptosis induction were also observed in these cell lines.
Antiproliferative activity
Cell proliferation was determined using the 3-(4,5-dimethylthiazol-2-yl)-2,5-diphenyltetrazolium bromide (MTT) assay. HepG2 and Hep3B cells were plated at a density of (2.5-5) ×105 cells/well in 96-well tissue culture plate (Corning, NY, USA), and incubated at 37 °C for 24 h. Plated cells were treated with APME ranging from 5 to 200 µg/mL. After incubation for 24, 48, and 72 h, plated cells were incubated with MTT (Sigma-Aldrich Co., LLC., CA, USA, 0.5 mg/mL final concentration) for 4 h at 37 °C. After discarding the medium from the plates, 100 µL of DMSO was added to each well. The plates were placed for 5 min at room temperature with shaking, so that complete dissolution of formazan was achieved. The absorbance of the MTT formazan was determined at 540 nm by a UV/VIS spectrophotometric plate reader (Emax; MolecularDevices). The IC50 value, the concentration of the extract required to inhibit cancer cell growth by 50% of the control level, was estimated from the plot.
Cell cycle distribution
Cells were then harvested, washed with cold PBS, and processed for cell cycle analysis. Briefly, the cells were fixed in absolute ethanol and stored at –20 °C for later analysis. The fixed cells were centrifuged at 1,000 r/min and washed with cold PBS twice. RNase A (20 µg/mL final concentration) and propidium iodide staining solution (50 µg/mL final concentration) were added to the cells and incubated for 30 min at 37 °C in the dark. The cells were analyzed by a FACS Calibur instrument (BD Biosciences, San Jose, CA, USA) equipped with CellQuest 3.3 software. ModFit LT 3.1 trial cell cycle analysis software was used to determine the percentage of cells in the different phases of the cell cycle.
Apoptotic features
Apoptotic features of HepG2 and Hep3B cells exposed to APME were observed using a Nikon inverse phase contrast microscope (Nikon TMS, Nikon, Japan) equipped with an objective (Plan 10/0.30DL/Ph1, Nikon) of ×100 magnification.
P53, Bcl-2 and Bax expression
Protein expression was determined by Western blotting. Briefly, cells were lysed in RIPA buffer (1% NP-40, 150 mmol/L NaCl, 0.05% DOC, 1% SDS, 50 mmol/L Tris, pH 7.5) containing protease inhibitor for 1 h at 4 °C. The supernatant was separated by centrifugation, and protein concentration was determined by Bradford protein assay kit II (Bio-rad Laboratories, CA, USA). For detection of cytochrome c release, cytosolic fraction was sedimented from post-nuclear supernatant by centrifugation at 100,000 g for 30 min at 4 °C. Proteins (25 µg/well) denatured with sample buffer were separated by 10% SDS-polyacrylamide gel (Bio-rad Laboratories, CA, USA). Proteins were transferred onto nitrocellulose membranes (0.45 µm). The membranes were blocked with 1% bovine serum albumin (BSA) solution for 1.5 h and washed twice with PBS containing 0.2% Tween-20, and incubated with the respective primary antibodies P53, Bcl-2, Bax, and β-actin (Cell Signaling Technology, Inc., Danvers, CA, USA) overnight at 4 °C. The next day, the immunoreaction was continued with the secondary goat anti-rabbit horseradish-peroxidase-conjugated antibody (Santa Cruz Biotechnology, Inc., CA, USA) after washing for 2 h at room temperature. The specific protein bands were detected by Opti-4CN Substrate kit (Bio-rad Laboratories, CA, USA).
Statistical analyses
All of the experiments were repeated four times. The data are presented as the x̄±s (n=4-7). The ROS scavenging and antiproliferative activity data are expressed as percentage compared with vehicle-treated control cells, which were arbitrarily assigned 100%. Data were analyzed by one-way analysis of variance followed by Dunnett’s multiple comparison tests (SigmaStat, Jandel, San Rafael, CA, USA). For all comparisons, differences were considered statistically significant at P<0.05.
Results
Antioxidant activity of APME
To investigate the antioxidant activity of APME in vitro, the HepG2 cell line, which was derived from a hepatocellular carcinoma, was used because it retains many of the characteristics of normal hepatocytes including phase I, phase II, and the expression of antioxidant enzymes (9-11).
APME showed a ROS scavenging ability in HepG2 cells (Figure 1). ROS generation was significantly increased in HepG2 cells in the presence of H2O2 as compared with control levels (2.6- and 2.0-fold for DHR123 and H2DCFDA, respectively, Figure 1A). At a concentration of 200 µg/mL, APME reduced the generation of ROS compared with the control group (by 53.7% and 49.4% for DHR123 and H2DCFDA, respectively). Additionally, APME scavenged ROS in all treatment groups in the absence of H2O2 (10-15% decrease, Figure 1B), but the difference was not statistically significant.
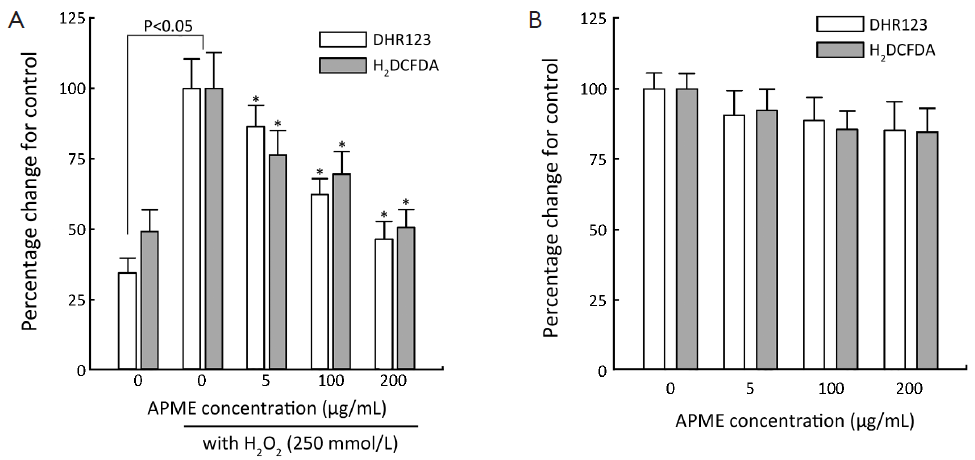
APME significantly increased the activity of antioxidant enzymes SOD and catalase at higher concentrations (Figure 2). APME increased catalase activity by 18.0% and 23.7% at concentrations of 100 and 200 µg/mL, respectively, compared with control levels. Total SOD activity was increased by 21.7% in cells treated with 200 µg/mL APME.
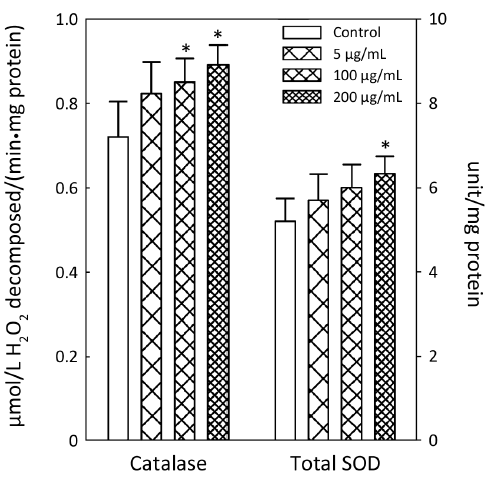
Anticancer activity of APME
The antiproliferative effect of APME in HepG2 and Hep3B cells exposed to various concentrations of APME (5, 10, 25, 50, 75, 100, 125, 150, and 200 µg/mL) for 24, 48, and 72 h was determined by MTT assay (Figure 3). APME reduced cell proliferation in a dose- and time-dependent manner (P<0.05). Inhibition increased significantly after 48 and 72 h in both HepG2 and Hep3B cells. HepG2 cells were more sensitive to APME treatment; a high concentration of APME (200 µg/mL) inhibited the proliferation of HepG2 and Hep3B cells by 36.5% and 26.2%, respectively, compared with control levels.
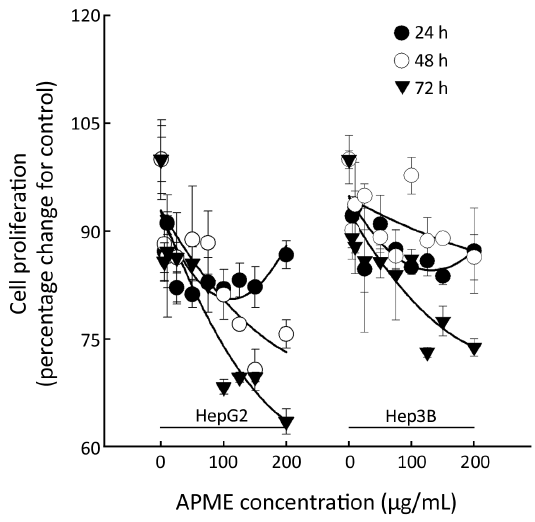
Next, HepG2 and Hep3B cells were treated with 50, 150, and 200 µg/mL APME for 72 h and analyzed for DNA synthesis arrest by fluorescence activated cell sorting (FACS) analysis (Figure 4). APME induced cell cycle arrest at the G1/S and G2/M phases in both cell lines in a dose-dependent manner. After exposure of HepG2 and Hep3B cells to a higher concentration of APME (200 µg/mL), the proportion of G1 phase cells increased by 28.9% and 14.6%, respectively, compared with control levels, and the proportion of G2/M phase cells decreased by 57.8% and 54.9%, respectively. APME also induced well-known apoptotic morphological features including membrane blebbing (Figure 5A,B).
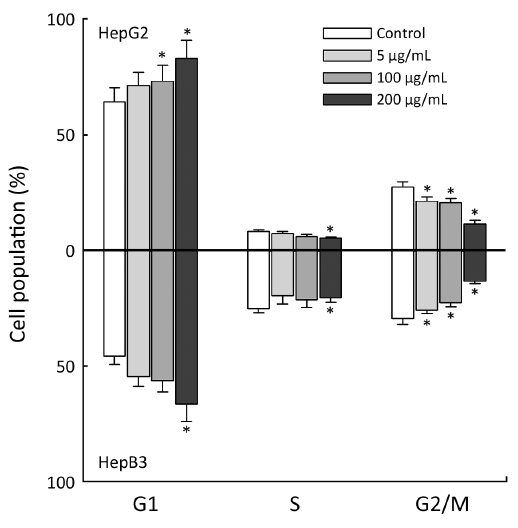
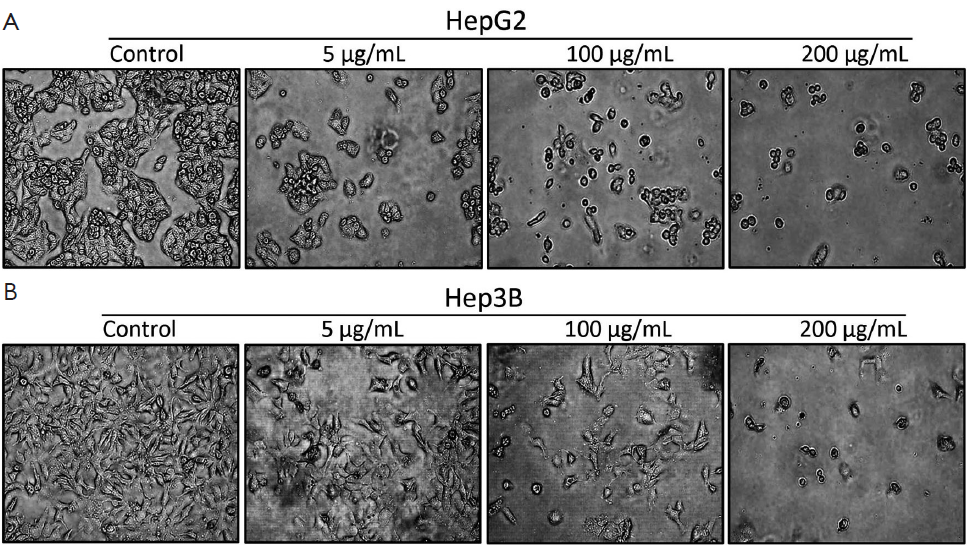
Moreover, the exposure of HepG2 cells to APME (5-100 µmol/L) for 72 h induced P53 expression of HepG2 cells in a dose-dependent manner (Figure 6A). In addition, Bcl-2 decreased while Bax increased in both HepG2 and Hep3B cells after exposure to APME (Figure 6A,B).
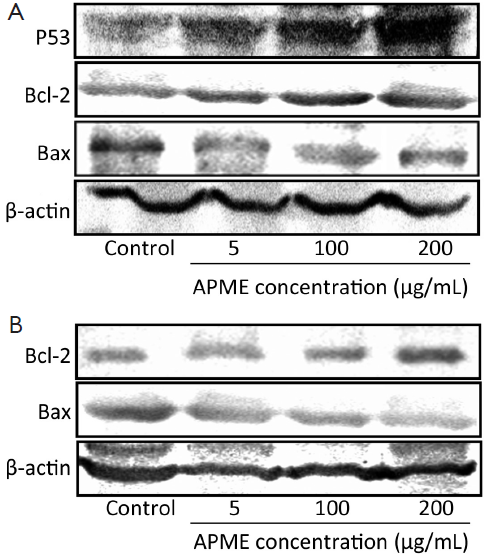
Discussion
The antioxidant activities of natural herb plants were thoroughly investigated long ago. Also, their crucial effects on the pathophysiology associated with cancers have recently received special attention. Due to many epidemiological reports suggesting that high consumption of antioxidant-rich plants significantly reduces the risk of many cancers (12,13), consumption of food supplement prepared from natural plants, has been highly recommended. Although numerous Artemisia species are used in folk medicine and pharmacies, scientific evidence of their effects is needed.
In the present study, the potential of APME as an antioxidant and anticancerigen preparation was evaluated. APME dose-dependently reduced ROS generation in the presence of hydrogen peroxide in HepG2 cells. Oxidative stress leads to an increase of ROS levels and a decrease of the levels of antioxidant molecules and enzymes. Cellular oxidative stress has been implicated in the etiology and pathology of many diseases. Additionally, we have demonstrated that high concentration of APME increases catalase and SOD activity by more than 20%. Among the antioxidant defense systems, SOD is the first and most important line of enzymatic defence against oxidative stress, particularly oxygen radicals. SOD scavenges superoxide by converting it to peroxide. Peroxide, in turn, is destroyed by catalase, which is widely distributed in all animal tissues. SOD and catalase act in a mutually supportive way with antioxidant enzymes to protect against ROS. This finding is in agreement with the results of previous studies, which found that the antioxidant effects of Artemisia species include free radical scavenging and promotion of cellular defense activity (14,15). Thus, APME may act as an antioxidant in HepG2 cells by scavenging ROS and stimulating catalase and SOD activity.
Unfortunately, our in vitro study did not include positive controls. However, we tested several classical antioxidant, such as Trolox (a derivative of α-tocopherol), in our preliminary study. When cells were exposed to 0.1-10 µmol/L Trolox in the presence of H2O2, Trolox scavenged significant amounts of ROS, even at 0.1 µmol/L (approximately 24% reduction to compare control levels). In the 10 µmol/L Trolox treatment, the ROS induction by H2O2 was nearly reduced to normal levels. Although APME had weak ROS scavenging activity compared with classical antioxidant Trolox, different functions of APME, such as its anticancer activity, may make up for this.
In both HepG2 (wild p53) and Hep3B (p53 null) cells, APME significantly inhibited cell proliferation in a dose- and time-dependent manner, but at concentrations lower than 100 µg/mL, the inhibition was less dose-dependent than time-dependent.
The antiproliferative activity of APME was stronger in HepG2 cells, which express functional P53. Generally, HepG2 cells are highly susceptible to chemical agent and drugs, while Hep3B cells are more resistant because of p53 deficiency (16,17). APME also increased P53 expression of HepG2 in a dose-dependent manner, and this is consistent with reports stating that Artemisia spp. showed induction of P53-mediated G1 phase arrest in A172 human neuroblastoma cells (18). However, APME decreased the Bcl-2 expression, while increasing Bax in both HepG2 and Hep3B cells. Members of the Bcl-2 family of proteins are critical regulators of the apoptotic pathway and include the major anti-apoptotic family members Bcl-2 and Bcl-X(L) and the major pro-apoptotic proteins Bax and Bak. Thus, the anticancer mechanism of APME may involve both P53-dependent and -independent pathways. To further scrutinize these results, we analyzed the cell cycle distribution using FACS and observed features of apoptosis in HepG2 and Hep3B cells exposed to 5, 100, and 200 µg/mL APME for 72 h. Cell cycle arrest was not induced in cells exposed to low concentration of APME, but significant G1 and G2/M phase arrest was induced at higher APME concentrations (100 and 200 µg/mL). Cell cycle arrest plays a pivotal role in tumor progression. Many reports indicate that various phytochemicals induce cell cycle arrest in human cancer cells, and that Artemisia species inhibit the cell cycle at the G1 or G2/M phase in various types of human cancer cells (19-23). Thus, APME may act as an anticancer preparation in HepG2 and Hep3B cells by inhibiting cell growth and arresting the cell cycle.
The use of products from Artemisia species, as important medicines for human health, illustrates the health potential of natural plants. In the present study, APME showed significant antioxidant and anticancer activity in hepatocarcinoma cell lines.
Acknowledgements
This work was supported by Priority Research Centers Program through the National Research Foundation of Korea (NRF) funded by the Ministry of Education, Science and Technology (NRF-2009-0094017 and 2011-0017017).
Disclosure: The authors declare no conflict of interest.
References
- Kim SH, Kim SH, Lee SD, et al. Determination of a new antiulcer agent, eupatilin, in rat plasma, bile, urine, and liver homogenate by high-performance liquid chromatography. Res Commun Mol Pathol Pharmacol 1997;97:165-70. [PubMed]
- Ryu SY, Kim JO, Choi SU. Cytotoxic components of Artemisia princeps. Planta Med 1997;63:384-5. [PubMed]
- Wink M. Production and application of phytochemicals from an agricultural perspective. In: vanBeek TA, Breteler H. eds. Phytochemistry and Agriculture. Oxford: Clarendon, 1993;171-213.
- Tan RX, Zheng WF, Tang HQ. Biologically active substances from the genus Artemisia. Planta Med 1998;64:295-302. [PubMed]
- Huang CF, Lin SS, Liao PH, et al. The immunopharmaceutical effects and mechanisms of herb medicine. Cell Mol Immunol 2008;5:23-31. [PubMed]
- Sarath VJ, So CS, Won YD, et al. Artemisia princeps var orientalis induces apoptosis in human breast cancer MCF-7 cells. Anticancer Res 2007;27:3891-8. [PubMed]
- Aebi H. Catalase in vitro. Methods Enzymol 1984;105:121-6. [PubMed]
- Marklund S, Marklund G. Involvement of the superoxide anion radical in the autoxidation of pyrogallol and a convenient assay for superoxide dismutase. Eur J Biochem 1974;47:469-74. [PubMed]
- Zhang R, Sun J, Ma L, et al. Induction of cytochromes P450 1A1 and 1A2 by tanshinones in human HepG2 hepatoma cell line. Toxicol Appl Pharmacol 2011;252:18-27. [PubMed]
- Knasmüller S, Mersch-Sundermann V, Kevekordes S, et al. Use of human-derived liver cell lines for the detection of environmental and dietary genotoxicants; current state of knowledge. Toxicology 2004;198:315-28. [PubMed]
- Zhu XG, Ort DR, Whitmarsh J, et al. The slow reversibility of photosystem II thermal energy dissipation on transfer from high to low light may cause large losses in carbon gain by crop canopies: a theoretical analysis. J Exp Bot 2004;55:1167-75. [PubMed]
- Lee IM. Antioxidant vitamins in the prevention of cancer. Proc Assoc Am Physicians 1999;111:10-5. [PubMed]
- Machlin LJ. Critical assessment of the epidemiological data concerning the impact of antioxidant nutrients on cancer and cardiovascular disease. Crit Rev Food Sci Nutr 1995;35:41-50. [PubMed]
- Bora KS, Sharma A. Evaluation of antioxidant and free-radical scavenging potential of Artemisia absinthium. Pharm Biol 2011;49:1216-23. [PubMed]
- Kim KS, Lee S, Lee YS, et al. Anti-oxidant activities of the extracts from the herbs of Artemisia apiacea. J Ethnopharmacol 2003;85:69-72. [PubMed]
- Chi TY, Chen GG, Lai PB. Eicosapentaenoic acid induces Fas-mediated apoptosis through a p53-dependent pathway in hepatoma cells. Cancer J 2004;10:190-200. [PubMed]
- Murakami Y, Hayashi K, Hirohashi S, et al. Aberrations of the tumor suppressor p53 and retinoblastoma genes in human hepatocellular carcinomas. Cancer Res 1991;51:5520-25. [PubMed]
- Park EY, Lee KW, Lee HW, et al. The ethanol extract from Artemisia princeps Pampanini induces p53-mediated G1 phase arrest in A172 human neuroblastoma cells. J Med Food 2008;11:237-45. [PubMed]
- Cha JD, Moon SE, Kim HY, et al. Essential oil of Artemisia capillaris induces apoptosis in KB cells via mitochondrial stress and caspase activation mediated by MAPK-stimulated signaling pathway. J Food Sci 2009;74:T75-81. [PubMed]
- Hu YQ, Tan RX, Chu MY, et al. Apoptosis in human hepatoma cell line SMMC-7721 induced by water-soluble macromolecular components of Artemisia capillaris Thunberg. Jpn J Cancer Res 2000;91:113-7. [PubMed]
- Park EY, Lee KW, Lee HW, et al. The ethanol extract from Artemisia princeps Pampanini induces p53-mediated G1 phase arrest in A172 human neuroblastoma cells. J Med Food 2008;11:237-45. [PubMed]
- Koo HN, Hong SH, Jeong HJ, et al. Inhibitory effect of Artemisia capillaris on ethanol-induced cytokines (TNF-alpha, IL-1alpha) secretion in Hep G2 cells. Immunopharmacol Immunotoxicol 2002;24:441-53. [PubMed]
- Hong JH, Lee IS. Cytoprotective effect of Artemisia capillaris fractions on oxidative stress-induced apoptosis in V79 cells. Biofactors 2009;35:380-8. [PubMed]